Recreating Big Bang matter on Earth
By: Ana Lopes
13 NOVEMBER, 2020 · Voir en français
Our fifth story in the LHC Physics at Ten series looks at how the LHC has recreated and greatly advanced our knowledge of the state of matter that is believed to have existed shortly after the Big Bang
The Large Hadron Collider (LHC) at CERN usually collides protons together. It is these proton–proton collisions that led to the discovery of the Higgs boson in 2012. But the world’s biggest accelerator was also designed to smash together heavy ions, primarily the nuclei of lead atoms, and it does so every year for about one month. And for at least two good reasons. First, heavy-ion collisions at the LHC recreate in laboratory conditions the plasma of quarks and gluons that is thought to have existed shortly after the Big Bang. Second, the collisions can be used to test and study, at the highest manmade temperatures and densities, fundamental predictions of quantum chromodynamics, the theory of the strong force that binds quarks and gluons together into protons and neutrons and ultimately all atomic nuclei.
The LHC wasn’t the first machine to recreate Big Bang matter: back in 2000, experiments at the Super Proton Synchrotron at CERN found compelling evidence of the quark–gluon plasma. About five years later, experiments at the Relativistic Heavy Ion Collider (RHIC) at Brookhaven National Laboratory in the US started an era of detailed investigation of the quark–gluon plasma. However, in the 10 years since it achieved collisions at higher energies than its predecessors, the LHC has taken studies of the quark–gluon plasma to incredible new heights. By producing a hotter, denser and longer-lived quark–gluon plasma as well as a larger number and assortment of particles with which to probe its properties and effects, the LHC has allowed physicists to study the quark–gluon plasma with an unprecedented level of detail. What’s more, the machine has delivered some surprising results along the way, stimulating new theoretical studies of this state of matter.
“In the ultimate textbook about the theory of the strong interaction, the chapter on the quark–gluon plasma will be filled with figures of LHC data,” says ALICE experiment spokesperson Luciano Musa.
“These figures excel in data precision and kinematic reach, and they are the first to inform us about how quark–gluon plasma-like properties emerge gradually as one transitions from proton–proton to heavy-ion collisions.”
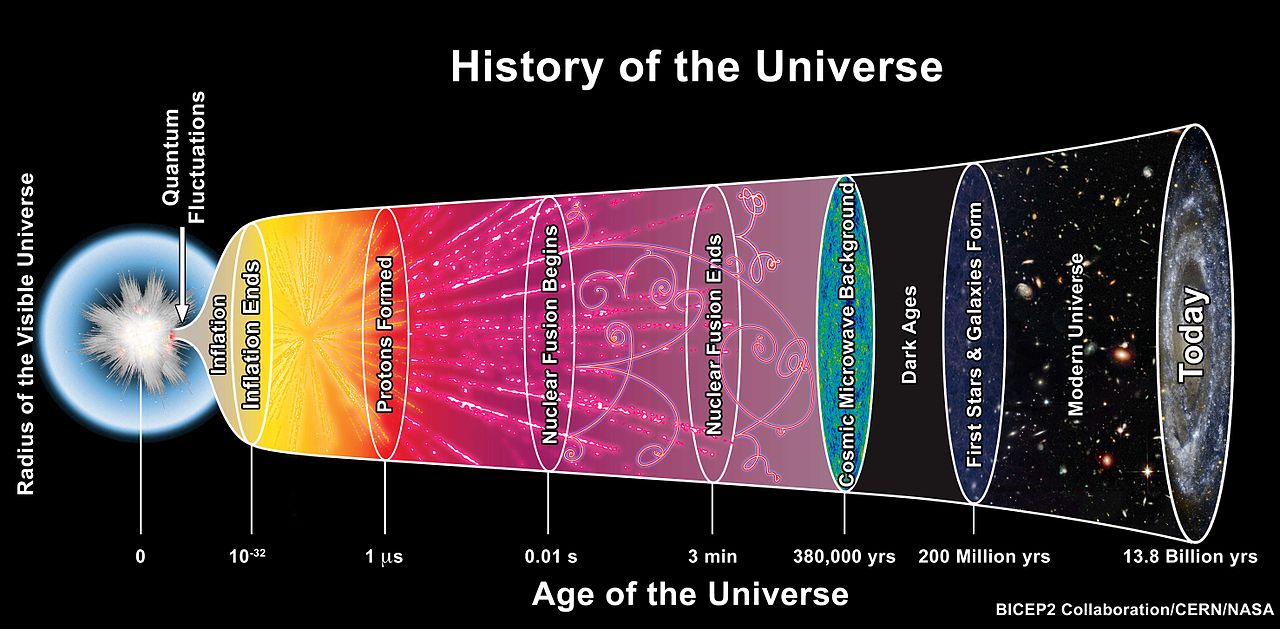
Heavy collision course
When heavy nuclei smash into one another in the LHC, the hundreds of protons and neutrons that make up the nuclei release a large fraction of their energy into a tiny volume, creating a fireball of quarks and gluons. These tiny bits of quark–gluon plasma only exist for fleeting moments, with the individual quarks and gluons, collectively known as partons, quickly forming composite particles and antiparticles that fly out in all directions. By studying the zoo of particles produced in the collisions – before, during and after the plasma is created – researchers can study the plasma from the moment it is produced to the moment it cools down and gives way to a state in which composite particles called hadrons can form. However, the plasma cannot be observed directly. Its presence and properties are deduced from the experimental signatures it leaves on the particles that are produced in the collisions and their comparison with theoretical models.
Such studies can be divided into two distinct categories. The first kind of study investigates the thousands of particles that emerge from a heavy-ion collision collectively, providing information about the global, macroscopic properties of the quark-gluon plasma. The second kind focuses on various types of particle with large mass or momentum, which are produced more rarely and offer a window into the inner, microscopic workings of the medium.
At the LHC, these studies are conducted by the collaborations behind all four main LHC experiments: ALICE, ATLAS, CMS and LHCb. Although ALICE was initially specifically designed to investigate the quark–gluon plasma, the other three experiments have also since joined this investigation.
Global properties
The LHC has delivered data that has enabled researchers to derive with higher precision than previously achieved several global properties of the medium.
“The LHC can “hear” much more precisely the quark–gluon plasma,” says CERN theorist and quark–gluon plasma specialist Urs Wiedemann.
“If we listen to two different musical instruments with closed eyes, we can distinguish between the instruments even when they are playing the same note. The reason is that a note comes with a set of overtones that give the instrument a unique distinct sound. This is but one example of how simple but powerful overtones are in identifying material properties. Heavy-ion physicists have learnt how to make use of “overtones” in their study of the quark–gluon plasma. The initial stage of a heavy-ion collision produces ripples in the plasma that travel through the medium and excite overtones. Such overtones can be measured by analysing the collective flow of particles that fly out of the plasma and reach the detectors. While previous measurements had revealed only first indications of these overtones, the LHC experiments have mapped them out in detail. Combined with other strides in precision, these data have been used by theorists to characterise the plasma’s properties, such as its temperature, energy density and frictional resistance, which is smaller than that of any other known fluid,” explains Wiedemann.
These findings have then been supported in multiple ways. For instance, the ALICE collaboration estimated the temperature of the plasma by studying photons that are emitted by the hot fireball. The estimated temperature, about 300 MeV (1 MeV is about 1010 kelvin), is above the predicted temperature necessary for the plasma to be created (about 160 MeV), and is about 40% higher than the one obtained by the RHIC collider.
Another example is the estimation of the energy density of the plasma in the initial stage of the collisions. ALICE and CMS obtained a value in the range 12–14 GeV per cubic femtometre (1 femtometre is 10-15 metres), about 2–3 times higher than that determined by RHIC, and again above the predicted energy density needed for the plasma to form (about 1 GeV/fm3).
Inner workings
The LHC has supplied not just more particles but also more varied types of particle with which to probe the quark–gluon plasma.
“The LHC has given us access to a very broad palette of probes,” says ALICE physics coordinator Andrea Dainese.
“Together with state-of-the-art particle detectors that cover more area around the collision points as well as sophisticated methods of identifying and tracking particles, this broad palette has offered unprecedented insight into the inner workings and effects of the quark–gluon plasma.”
To give a few examples, soon after the LHC started, ATLAS and CMS made the first direct observation of the phenomenon of jet quenching, in which jets of particles formed in the collisions lose energy as they cross the quark–gluon plasma medium. The collaborations found a striking imbalance in the energies of pairs of jets, with one jet almost completely absorbed by the medium.
Another example concerns heavy quarks. Such particles are excellent probes of the quark–gluon plasma because they are produced in the initial stages of a heavy-ion collision and therefore experience the entire evolution of the plasma. The ALICE collaboration has more recently shown that heavy quarks “feel” the shape and size of the quark–gluon plasma, indicating that even the heaviest quarks move with the medium, which is mostly made of light quarks and gluons.
The LHC experiments, in particular ALICE and CMS, have also significantly improved our understanding of the hierarchical “melting” in the plasma of bound states of a heavy quark and its antiquark, called quarkonia. The more weakly bound the states are, the more easily they will melt, and as a result the less abundant they will be. CMS was the first to observe this so-called hierarchical suppression for bottomonium states, which consist of a bottom quark and its antiquark. And ALICE revealed that, while the most common form of charmonium states, which are composed of a charm quark and its antiquark, is highly suppressed due to the effect of the plasma, it is also regenerated by the recombination of charm quarks and antiquarks. This recombination phenomenon, observed for the first time at the LHC, provides an important testing ground for theoretical models and phenomenology, which forms a link between the theoretical models and experimental data.
Surprises in smaller systems
The LHC data have also revealed unexpected results. For example, the ALICE collaboration showed that the enhanced production of strange hadrons (particles containing at least one strange quark), which is traditionally viewed as a signature of the quark-gluon plasma, arises gradually in proton–proton and proton–lead collisions as the number of particles produced in the collisions, or “multiplicity”, increases.
Another case in point is the gradual onset of a flow-like feature with the shape of a ridge with increasing multiplicity, which was first observed by CMS in proton–proton and proton–lead collisions. This result was further supported by ALICE and ATLAS observations of the emergence of double-ridge features in proton–lead collisions.
“The LHC data have killed the long-held view that proton–proton collisions produce free-streaming sets of particles while heavy-ion collisions produce a fully developed quark–gluon plasma. And they tell us that in the small proton–proton collision systems there are more physical mechanisms at work than traditionally thought. The new challenge is to understand, within the theory of the strong force, how quark–gluon plasma-like properties emerge gradually with the size of the collision system.”
These are just examples of how 10 years of the LHC have greatly advanced physicists’ knowledge of the quark–gluon plasma and thus of the early universe. And with data from the machine’s second run still being analysed and more data to come from the next run and the High-Luminosity LHC, the LHC’s successor, an even more detailed understanding of this unique state of matter is bound to emerge, perhaps with new surprises in the mix.
“The coming decade at the LHC offers many opportunities for further exploration of the quark–gluon plasma,” says Musa. “The expected tenfold increase in the number of lead–lead collisions should both increase the precision of measurements of known probes of the medium and give us access to new probes. In addition, we plan to explore collisions between lighter nuclei, which could cast further light on the nature of the medium.”
Further reading:
LHC at 10: the physics legacy
First Results from Pb+Pb collisions at the LHC
Heavy-ion collisions at the LHC
Heavy-ion collisions at the Large Hadron Collider: a review of the results from Run 1
An overview of experimental results from ultra-relativistic heavy-ion collisions at the CERN LHC: bulk properties and dynamical evolution
An overview of experimental results from ultra-relativistic heavy-ion collisions at the CERN LHC: hard probes
Don't miss the next articles of our series, which will cover the Standard Model and more.